Bioplastics are a subject of increasing interest due to demand for traditional petroleum alternatives. Several pathways to bioplastic generation are known today
The most prominent are derived from plants, with plastic derived from starches making up just over 50 per cent of the market. This method depends on excess organic feedstocks including rice and corn that redirects valuable foods from human and animal consumers, and contributes to waste output due to unused resulting plant material as well as an increase in the demands of large-scale agriculture. Therefore, there is a need for alternative pathways to be improved, especially those that demonstrate as sustainable of a method as possible. This route may be found in bacteria: through microbial electrosynthesis and more traditional biosynthesis under sugar consumption, bacteria and archaea have been found to produce a host of metabolic end-products resulting from the consumption of CO2 and various organic waste products. Notable outcomes include ethanol, acetic acid, and bioplastics. The former two are well-characterized with a myriad of uses, most commonly as a fuel and preservative, respectively. Advances in bioplastic generation beg the consideration of which kinds of bioplastics are usable now, which kinds require additional research, and what methods are available to make bioplastics sustainable for long-term use.
Bioplastic generated by bacteria can take many forms falling under the polyhydroxyalkanoate (PHA) family. In most cases, PHAs, as extracted from cells, are undesirable and require further processing. Prone to easy breakdown and brittle, many unrefined PHAs do not seem to be a viable alternative to petroleum-derived plastics. However, increased generation of bacteria-sourced plastics can be developed using genetic engineering and post-production chemical processing. Various subsets of PHAs are described below.
PHB:
Poly-hydroxybutyrates or PHB is a bioplastic with properties similar to polyethylene (PE). PE is the most common plastic on earth and is largely used for single-use products. Therefore, PHB has the potential for a wide range of uses including containers and cutlery.
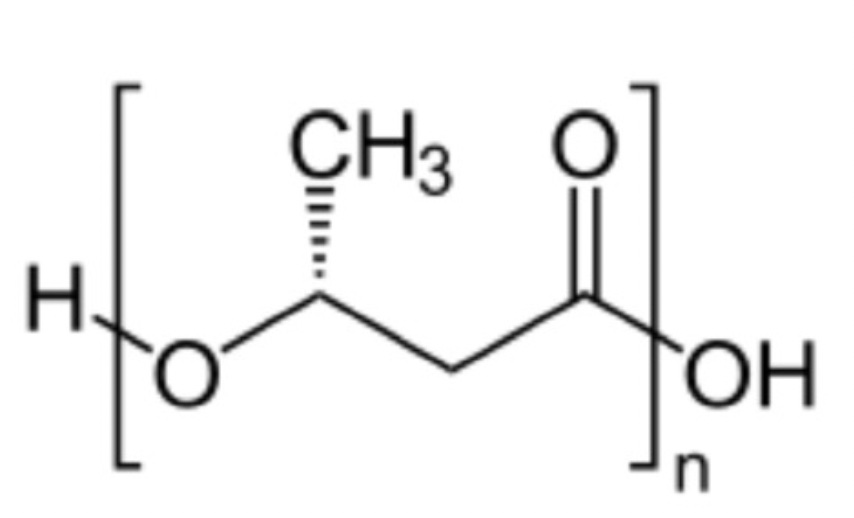
PHBV:
Poly(3-hydroxybutyrate-co-3-hydroxyvalerate). With different properties including increased porosity and flexibility compared to PHB, PHBV has uses in controlled releases of drugs, specialty packaging and other medical applications.
PHBHHx:
Poly(3-hydroxybutyrate-co-3- hydroxyhexanoate PHBHHx has the unique property of having good biocompatibility, meaning that it is able to be integrated into human systems with minimal rejection or harm to the host. Recent literature has explored its potential for tissue scaffolding.
Given the varied kinds of bioplastic uses and increasing investment into bioplastic development and generation, it is clear that not only is research into the production of bioplastic a worthwhile investment, but various means of degradation and bioremediation are and will continue to be critically important in the near future.
Bioplastic Generation: Source
Bacteria have a wide variety of biosynthetic pathways, granting them the abilities to produce complex molecular end- and by-products, as well as allowing versatility in methods to survive. Some methods and pathways require less energy on the part of the bacterium, and are the paths most often followed. Some pathways are rare: some bacteria receive genes through horizontal gene transfer or have evolved machinery to make new nutrients if the environment cannot provide any. A careful selection of both biosynthetic pathways and bacterial chassis species are the most important decisions in any bioplastic feasibility study where the goal is to develop a means of production that is reliable, sustainable, and affordable as a petroleum-based plastic alternative.
Heterotrophy:
The most widely studied and best understood means of bioplastic generation happens under heterotrophic growth, when essential molecules are consumed by bacteria directly rather than relying on their own machinery to create essential precursor molecules from base components. If a bacterium has access to a carbon and energy rich carbon-source, it will prefer this path over one that begins with a more difficult to assimilate starting material. This is the easiest way to grow large quantities of microbes and produce large quantities of product. However, it relies on a continued supply of sugars to produce bioplastics, and faces a major loss of environmental benefit compared to microbial electrosynthesis or some chemo- or autotrophic methods due to its lack of ability to remediate waste products or carbon dioxide during the growth and plastic production process.
Photoautotrophs and chemoutotrophs:
A photoautotroph contains the machinery needed to assimilate inorganic carbon using sunlight as an energy source, while a chemoautotroph oxidizes chemical substances in its environment to produce the necessary energy for inorganic carbon assimilation. One type of microorganism that may lend itself particularly well to sustainable biosynthesis is capable of performing photoferrotrophy, a photoautotrophic metabolism where bacteria oxidize iron to create bioplastics using carbon dioxide from the environment.
Because these organisms are capable of carbon dioxide bioremediation to produce bioplastics, they have an advantage over heterotrophs in that they don’t need organic feedstock, saving load on agriculture. However, these organisms still require chemicals, in this case iron, to thrive. To satisfy this need sustainably, it may be possible to look to the field of microbial electrosynthesis as a beneficial alternative.
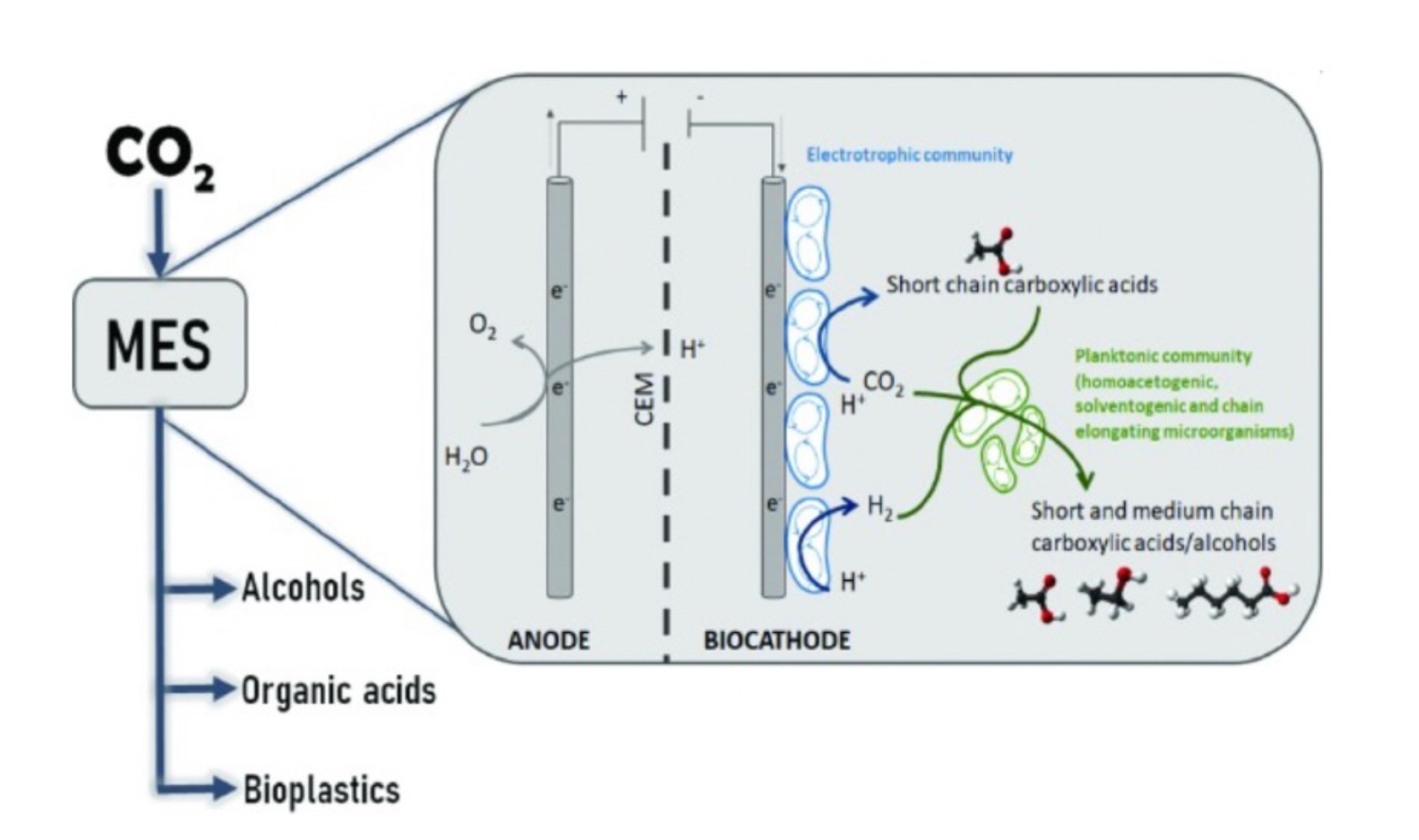
MES:
Microbial electrosynthesis (MES) is a new field of microbiology studying bacterial production of bioplastics and fuels using CO2 and electricity as inputs. It has huge potential as a greener and more sustainable alternative to heterotrophic and autotrophic pathways that require additional compounds to be added to media in order to grow.
MES operates through a set of redox reactions allowed to occur because bacteria can reduce carbon dioxide using electrons supplied by the electric current. In the above figure, a planktonic community and electrotrophic community operate in conjunction to reduce CO2 to short chain carboxylic acids, and then elongate those acids into larger chain products.
Other electrotrophic bacteria, like Rhodopseudomonas Palustris TIE-1, are able to do so without a consortium.
In either case, CO2 is consumed, which indicates a potential use for excess of this gas in our atmosphere and converted to organic products such as bioplastics. This joint set of benefits creates something called a closed carbon loop. In traditional fuel burning and plastic creation, petroleum is burned or degraded which releases “new” CO2 that had been sequestered underground for a geological timescale into the atmosphere; here, CO2 molecules from the atmosphere are recycled into bioproducts. This prevents the addition of previously-sequestered carbon into the cycle, leading to reduced negative downstream effects such as increased greenhouse gas (CO2) emissions. Despite the benefits outlined here, MES still has several major roadblocks that must be addressed before it can be reliably used for bioplastic generation at an industrial scale.
In an MES bioreactor, bacteria form biofilms on the surface of the cathode, meaning that biofouling becomes an issue. Biofouling occurs when mats of bacteria or bacterial products accumulate on surfaces, which can interfere with processes that rely on exchange with those surfaces, such as the electron exchange in MES bioreactors. In large scale reactors, thick mats of biofilm formation could reduce the efficiency of bacteria to form bioproducts. Although this is a field of study currently under investigation, much more insight into biofilm effects and mediation of MES is needed.
MES also faces the issue in that the electricity needed may produce greenhouse gas waste as a byproduct. Because microbes in such a system require a stream of electricity to supply essential electrons, industrial-scale systems may require a substantial amount of energy to operate. If the production of bioplastics uses electricity derived from fossil fuels, then carbon dioxide emissions still occur, and MES loses much of its sustainability benefit. Therefore, there have been proposals to set up systems using solar powered or other green energy sources to supply electricity.
Finally, there are other obstacles to obtaining bioplastics from microbes. The major one is bioplastic extraction from bacteria; to do so currently requires the addition of a lysing agent, such as a high-molarity acid, as well as an organic, like chloroform, to separate the cell material from the bioplastic. This process is simultaneously dangerous as well as difficult to navigate in an industrial setting due to the production of large volumes of hazardous waste. To make bioplastics a viable alternative to petroleum plastics, this is a major step that needs to be addressed.
Sustainable futures: Breakdown and Use
Having established that bioplastics offer a suite of exciting and specific applications, further thought is needed to determine the best methods of disposal. Like petroleum plastic that is not recycled and is instead placed in a landfill, a poorly considered method of disposal will circumvent much of the effort put into creating sustainable plastics.
Plant and microbe-generated bioplastics can be bioremediated, which involves the use of plastic-degrading bacteria that use lactic acid to degrade and consume starch-based plastics for consumption. But more studies are needed to assess how bacteria are able to consume PHAs. Because PHAs are produced as a reserve energy source inside the cells of some bacteria, it is hypothesized that they can be consumed and recycled by the same species of bacteria that produce them in the wild. However, because bacteria do not secrete the bioplastic they produce, studies surrounding chemical transformation of bioplastic or a bioplastic transporter that can bring plastic particles into the cell are needed.
Feedstock bioplastic degradation:
Contrary to bioplastic synthesis, there is no clear-cut way for zero waste biodegradation. Biodegradability is defined as the bioconversion of material by microorganisms and their enzymes into biomass, CO2, CH4, water and metabolites. Therefore, in a biodegradation system, there should be no negative byproducts such as microplastics or residual polymers that cannot be further metabolized by other organisms.
Taking this into account, the only currently feasible ways that are available for organic recycling of biodegradable materials are composting and anaerobic digestion. Composting, which is defined as the natural process of repurposing organic matter into fertilizer, has many benefits. For example, composting biodegradable material diverts it from landfills. Since organic waste produces large amounts of greenhouse gasses when there is a lack of oxygen (such as in a traditional capped landfill), composting has the potential to help reduce the effects of climate change. Additionally, composting also would decrease the production of fossil-based CO2 and generate biogenic CO2 which can then be repurposed into beneficial alternatives such as fertilizer. However, true composting for bioplastics is rare as facilities that have handled this process often do not follow the recommended amount of time needed for successful degradation. Aside from this, there are also other problems with composting. When usual biodegradable PCPP, or post-consumer plastic packaging, products such as polyhydroxyalkanoates (PHAs), poly(butylene adipate-co- terephthalate) (PBAT), and polylactic acid (PLA) etc. are composted, they mainly release CO2 and, therefore, contribute very little to soil nutrients and biogas recovery.
As for anaerobic digestion, it is a process that is still in its infancy since it requires much more background information about environmental conditions that may affect the efficiency of microbial activity. Anaerobic digestion is the decomposition of organic matter via bacteria in the absence of oxygen and, thus, is very applicable for bioplastic degradation. This process is significant as it can reduce plastic waste and recover bioenergy in the form of biogas due to the large carbon-to-nitrogen ratio. Biogas is a renewable energy source derived from the breakdown of organic matter, such as bioplastics. However, like composting, anaerobic digestion has its own faults as it would result in significant material loss and the creation of CO2 (when the biogas is burnt as fuel). In addition, many facilities that process organic waste through anaerobic digestion still consider biodegradable bioplastics as a contaminant due to the unknown impact of the generation of microplastics on the environment.
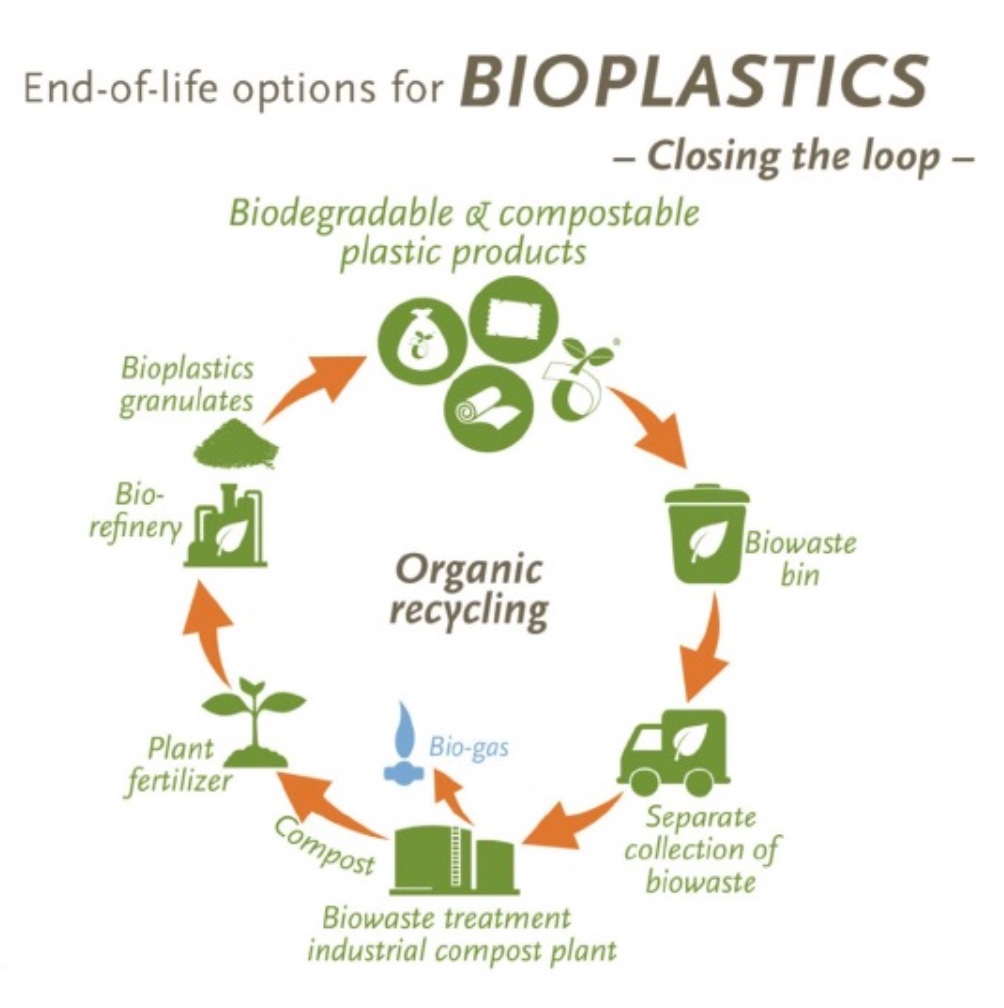
Degradation of all microbial plastics:
While the microbial creation of bioplastics is a major step forward in addressing the growing plastic problem, the key to a self-sustaining cycle of renewable materials is zero waste generation. One of the most promising ways to address this is using an organism’s natural biochemical processes, whether it is bacteria, algae, fungi etc., to degrade bioplastics into harmless products such as carbon dioxide and water. Therefore, the first step is to determine which specific species can degrade bioplastics naturally by using it as a carbon source. Once a species has been identified, it is also important to consider other factors that might affect the efficiency of degradation such as whether it can perform the degradation in the presence or absence of oxygen, the amount of bioplastic it is able to degrade, the temperature it prefers, and the pH constraints it needs. For example, the Bose Lab has identified that by using polyhydroxybutyrate (PHB) as a carbon source, Rhodopseudomonas palustris TIE-1 (TIE-1) has the ability to break down PHB in both aerobic and anaerobic conditions. Future studies can expand upon the efficiency of PHB degradation as well as establish if this process could be applicable at larger scales.
Beyond the general processes of identifying which organisms may have the ability to break down bioplastics, it is also important to note the mechanisms behind degradation. Various organisms may possess pathways that have co-evolved to break down the same exact starting material, but which may operate in entirely different ways using totally different enzymes. Furthermore, there are many bio-based or biodegradable bioplastics such as polyhydroxybutyrate (PHB) as one kind of polyhydroxyalkanoates (PHAs), poly(butylene adipate-coterephthalate) (PBAT), polybutylene succinate (PBS), polylactic acid (PLA), and polycaprolactone (PCL). They are made of polyester polymers composed of repeating monomer units bonded together by ester linkages. Therefore, these compounds can be degraded by hydrolysis of the ester bonds by esterases.
Specifically for PHB, there are also two different classifications of degradation: intracellular and extracellular. The most common and well-studied form is intracellular PHB degradation since there are many bacteria that have intracellular PHB depolymerases. Acidovorax sp., Acinetobacter sp., and Ochrobactrum sp. have been shown to degrade PHB extracellularly although the underlying mechanism is unclear. Discovering the mechanisms that allow PHB to be degraded extracellularly remains a challenge; however, since there are up to one trillion species of prokaryotes out in nature that have yet to be explored, we can predict the discovery of mechanisms of extracellular PHB degradation in promising new PHB-degrading bacteria in the future.
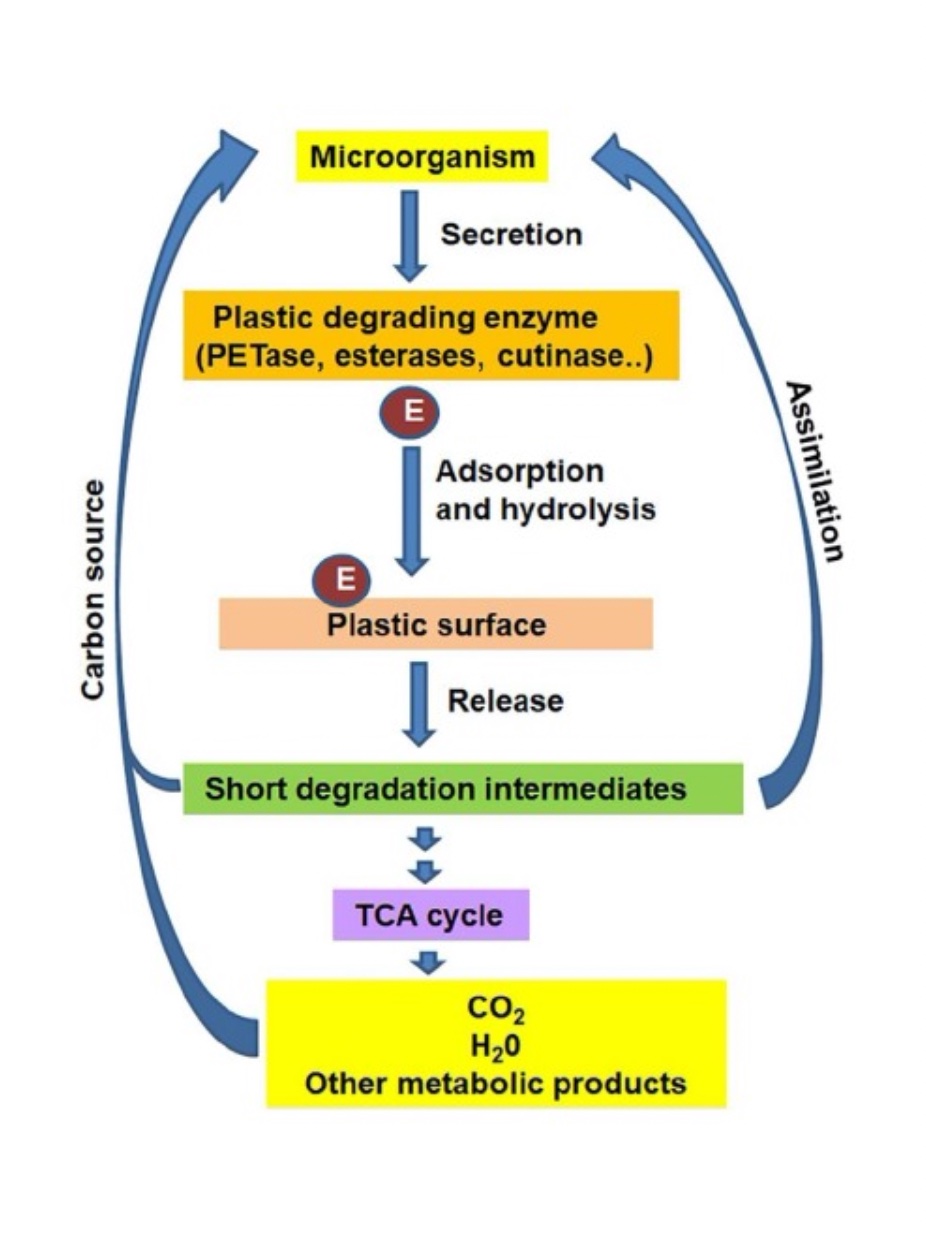
For more information and to download the full eBook here: ‘Bioplastics and back: Bacterial construction and degradation methods’