Bacteria are often painted as the enemy of humanity. Before the discovery of antibiotics, a wound getting infected was frequently a death sentence
Even with modern medicine, infections such as C. difficile and infectious diseases such as tuberculosis continue to kill many people globally. Crop blights and diseases among livestock cause extensive monetary losses and threaten food security in affected areas.
With all of these reasons to distrust bacteria, it may come as a surprise that certain microbes are immensely beneficial to humanity. Unfortunately, they rarely get to enjoy the limelight.
Our gut microbiomes help us digest complex molecules in our food, and many of the antibiotics we discovered were invented by microbes facing competition from their neighbors. We have leveraged microbes to process sewage and waste for centuries and to ferment food for millennia.
Certain microbes, which have the right metabolic pathways or would be a good chassis for expressing them, are likely to be one of our greatest assets in developing technologies to address climate change, environmental pollution, and the energy crisis.
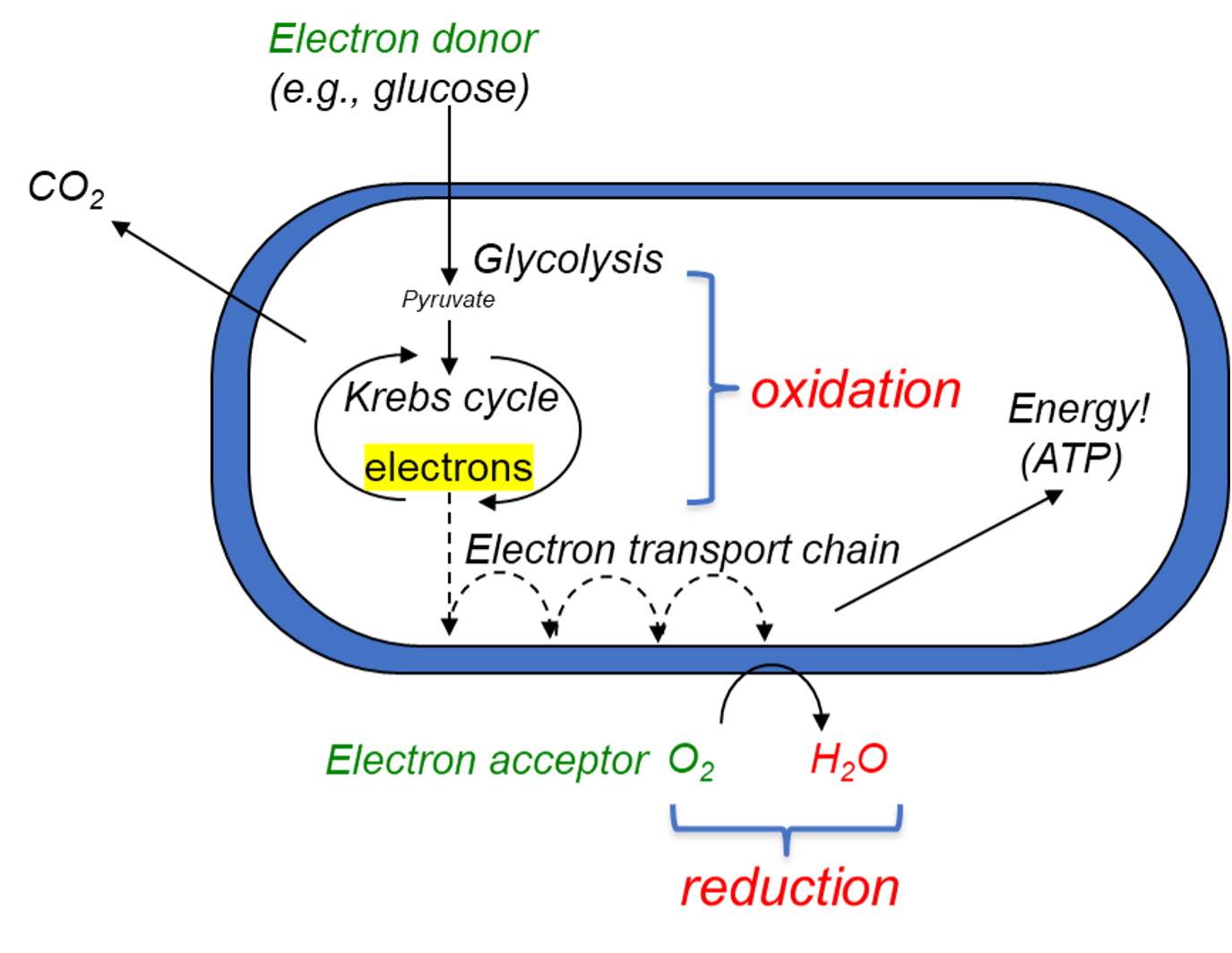
Microbial metabolism: oxidation and reduction
Living things keep living by producing energy through metabolism. Microbial metabolism primarily occurs through oxidation-reduction processes (redox reactions), exchanging electrons with their environment. Aerobic heterotrophs are bacteria with metabolisms similar to ours— they eat organic molecules (for example, glucose sugar) and breathe oxygen. The electrons used to make energy for these microbes come from their organic “food.”
Alternatively, many microbes acquire electrons from inorganic electron donors, including iron(II). Chemolithotrophs, like Mariprofundus ferrooxydans and Acidithiobacillus ferrooxidans, generate energy by oxidizing (or taking electrons from) soluble iron(II), using CO2 as a carbon source and producing insoluble iron(III) in the process. Photosynthetic iron-oxidizing organisms (photoferrotrophs) like Rhodopseudomonas palustris also oxidize soluble iron(II) to insoluble iron(III), though they use these electrons to drive photosynthesis and CO2 fixation.
Some microbes have evolved mechanisms to acquire electrons from solid electron donors, which includes iron-containing minerals and poised electrodes. This process, referred to as extracellular electron uptake (EEU), ensures cells survive when soluble electron donors are scarce. The organisms above, in addition to extracting electrons from soluble electron donors, can also use EEU to extract electrons from insoluble sources, including solid surfaces. For example, R. palustris and Mariprofundus can both extract electrons from iron minerals and poised electrodes.
Microbes’ ability to perform EEU has significant industrial and biotechnological implications. Iron-oxidizing microbial biofilms on exposed surfaces of metal-containing structures can lead to microbiologically influenced corrosion (MIC). On the other hand, biofilms grown on poised electrodes can facilitate a variety of biotechnological applications, from energy generation to bioplastic production. Acidithiobacillus, due to its tolerance of acidic conditions, could play a role in bioremediation of acid mine drainage.
Other genera of bacteria, such as Shewanella and Geobacter, can also perform an inverse of EEU, by “breathing” iron(III) instead of air. When soluble electron acceptors are scarce, these bacteria can use solid electron acceptors. Shewanella and Geobacter can reduce iron by using it (instead of something like oxygen) as a terminal electron acceptor in a process called extracellular electron transfer (EET). Other bacteria that can perform EET can use other minerals. As we will see in the next section, both EET- and EEU-capable microbes have their uses in the lab and in industry.
These bacteria and ones like them, composing a diverse collection across various taxonomic groups, are collectively called electroactive microorganisms. The very same electrically conductive proteins that these organisms use to transfer electrons to or from minerals in their habitats can be used to interface with electrically charged surfaces such as poised electrodes. Electroactive microbes are a key area of research by electromicrobiologists for their existing and potential applications for industry and the environment. Electrotrophs are able to cut out the middleman and get their electrons directly from electricity, such as that from an electrode. Electrogens, on the other hand, donate electrons to a mineral electron acceptor and can be made to produce electric current by providing a poised electrode as the sole electron acceptor. This is the fundamental principle upon which microbial fuel cells (MFCs) operate.
Applications for Electroactive Microbes
These tiny organisms have enormous potential for scientific and industrial applications. Microbial fuel cells, as mentioned, leverage bacteria to generate electricity. To that same end, anaerobic digesters use a community of bacteria and methanogenic (methane-producing) archaea to eat organic waste, like spent brewery yeast. They also produce methane gas— chemically identical to “natural gas”— to be burnt as fuel. As we move away from a fossil fuel-powered economy, alternative energy sources like these could help supply the planet’s growing energy demands into the future.
Another application is in bioremediation of contaminated land like superfund sites – by seeding these areas with electroactive bacteria found from the environment or genetically modified to the purpose, and feeding the bacteria with electricity from electrodes, it is possible to get them to neutralize dangerous environmental contaminants like petroleum spills or dissolved heavy metals like uranium.
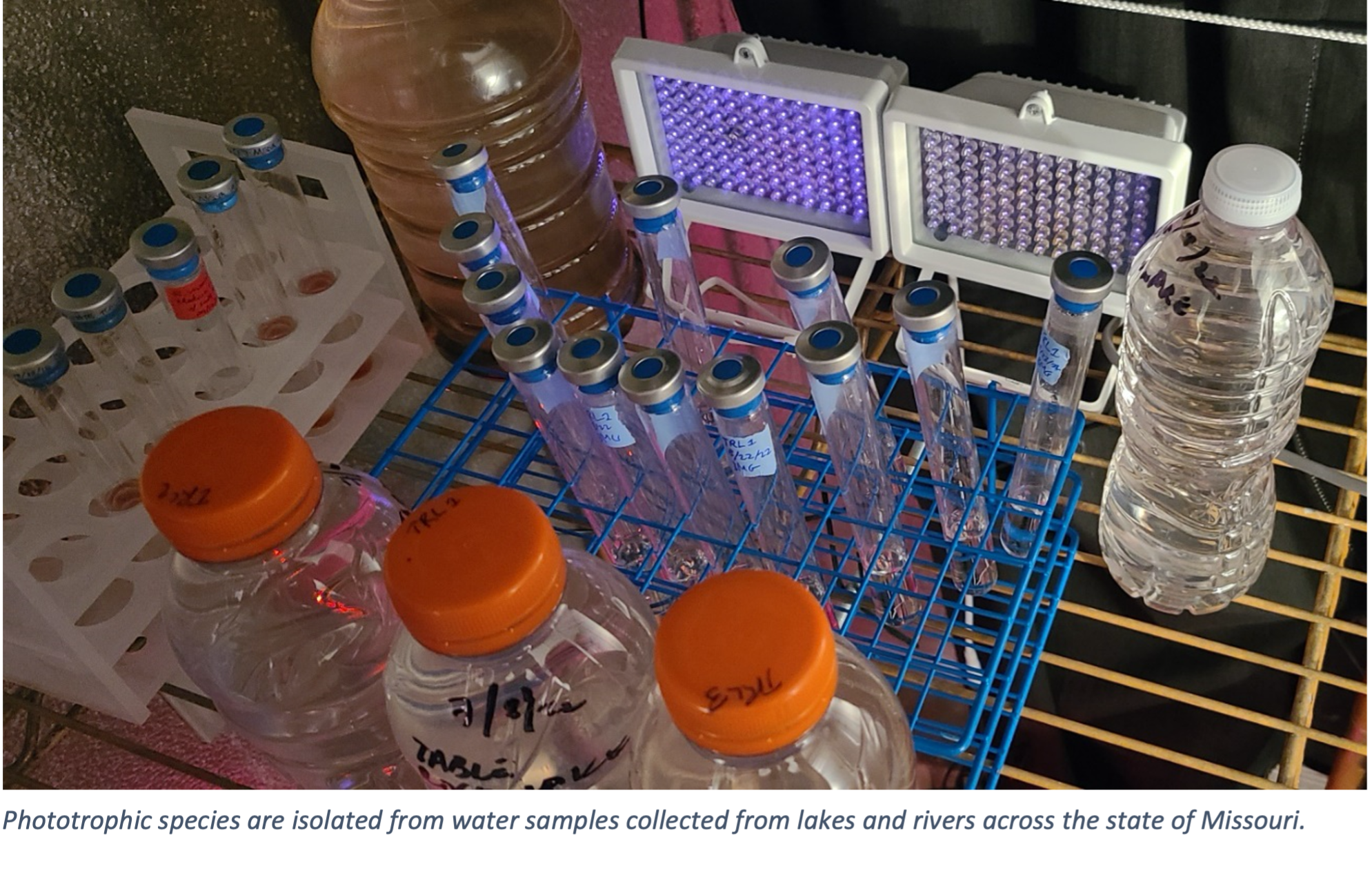
A third application, which shows some of the most promise for being rolled out at an industrial scale in the near future and is the major focus of the rest of this eBook, is microbial electrosynthesis.
Using Bacteria to Synthesize Organics
Biosynthesis is the process of using living organisms like bacteria or yeast to produce complex products from chemically simpler feedstock. Microbial Electrosynthesis (MES) refers to the process of using electroactive microbes to do this. MES comes with benefits over conventional biosynthesis, namely cost/efficiency of running the system— electricity is cheaper and easier to manage than organic electron sources.
Microbial synthesis of organics is already widely used in an industrial setting. For decades, we have used microorganisms to produce chemicals such as “alcohol, antibiotics, antioxidants, and natural colors.”
Rhodopseudomonas palustris TIE-1 (TIE-1) is a species of purple nonsulfur bacteria, named for its rosy color. This talented microbe is a veritable metabolic Swiss army knife, with various metabolic pathways in its back pocket that it can deploy depending on the situation. TIE-1 can use CO2 or organic carbon molecules as a food source, it can get its electrons from oxidizing iron through photoferrotrophy, and, to top it all off, it is capable of EEU from electrodes. Another characteristic, one that significantly advantages it as a chassis for MES applications, is that it is capable of performing anoxygenic (non-oxygen producing) photosynthesis to extract energy from sunlight.
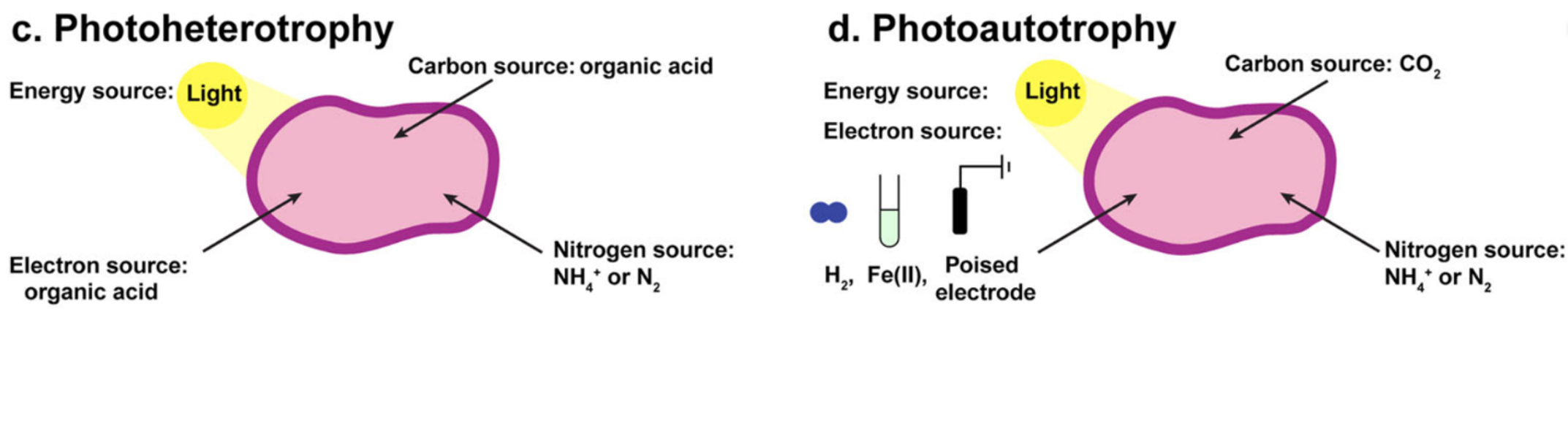
Purple nonsulfur bacteria like R. palustris can be found worldwide in oceans, rivers, lakes, and soils, as their flexible metabolism allows them to eke out a living in a wide variety of places. Their metabolic versatility, as well as their ability to perform photosynthesis for extra energy, makes them prime candidates for MES.
MES allows us to produce a wide range of organic products, but the most industrially relevant are biofuels and bioplastics. Today, the vast majority of plastic and fuel is made from fossil fuels extracted from the Earth— natural gas, oil, and coal. According to the Millennium Alliance for Humanity and the Biosphere (MAHB), a Stanford University initiative, these fossil fuels are running out: by 2100 the natural reserves will run dry if current rates of expenditure hold or increase. This is of course, only part of the problem— burning fossil fuels release their stored carbon back into circulation, increasing atmospheric CO2 levels and changing the climate, causing widespread ecological disasters, unpredictable weather events, and more.
Biofuels
In the United States, 95% of the gasoline we put in our cars contains 10% or more ethanol. Ethanol is the same type of alcohol found in bourbon and other liquors, and— like bourbon— the ethanol that is added to US gasoline is made primarily from corn, fermented by yeast and then distilled to concentrate it. The rationale for blending it with gasoline is twofold. First, ethanol produced this way is renewable, while petroleum is not. Second, burning it contributes less to climate change— USDA estimated that “greenhouse gas… emissions from corn ethanol are about 39 per cent lower than gasoline on an energy equivalent basis.” The primary issue with ethanol as a biofuel is that it directly competes with agriculture for the purpose of human nutrition, which is already an energy and land-intensive industry. Using corn-derived ethanol would cause even bigger issues if we were to switch to a higher percentage of ethanol in gasoline, such as the E85 (up to 83% ethanol) that can be burnt in flexible fuel vehicles. The energy contained in the fuel end product would need to be greater than the energy expended in growing, harvesting, and processing the corn for it to be worthwhile to change over to this biofuel from conventional fuels.
One of the best alternatives to ethanol is n- butanol, another type of alcohol that can be burnt as a biofuel but carries more energy per volume and is less volatile than ethanol. We typically produce it through chemical processes, though various microbes produce it naturally. Using a metabolic pathway called the acetone-butanol-ethanol (ABE) pathway, first discovered in Clostridium acetobutylicum, can allow microbes to ferment organic feedstock (ethanol or propylene) into n-butanol. This pathway has been engineered into other microbes, like E. coli and yeast, but because these organisms are heterotrophs, they still need to be fed organic carbon, running into the same problems as corn fermentation (namely, energy inefficiencies and the use of agricultural resources on the production of biofuels rather than food).
Clostridium, the organism the pathway was discovered in, is a relatively inefficient producer of n-butanol, so researchers began looking for an alternative microbial chassis for the pathway. Using photoautotrophs is more desirable as they can make use of sunlight, our most renewable energy resource. Synechococcus, a cyanobacterium (some of the first oxygen-producing phototrophs and the ancestors of chloroplasts), performed better than Clostridium when the ABE pathway was engineered into it, but there were concerns that the oxygen it produced during photosynthesis would negatively affect the process. This is where microbial electrosynthesis comes in. Here, EEU- capable microbes can produce products like n-butanol under anoxic conditions from CO2 and electrons produced by clean energy, rather than relying on costly organic feedstock to fuel the process.
Researchers in the Bose lab at Washington University in St Louis followed up on this tactic, by choosing the anoxygenic photoautotroph R. palustris TIE-1 as a chassis for n-butanol biosynthesis. This also allowed usage of light as an energy source, CO2 as a carbon source, and electrodes supplied with solar panel-generated electricity, making the biofuel production carbon neutral. Using this or a similar MES platform, industrial-scale bioreactors could produce carbon-neutral biofuel from renewable resources.
Bioplastics
Conventional petroleum-based plastics are a pernicious environmental issue, causing pollution both in the form of greenhouse gases during its production and as solid pollution in the form of plastic litter and microplastics, especially in the oceans. Even when disposed of properly and sent to a landfill (only around 20% gets recycled), many forms of plastic can last for the better part of a millennium.
Materials science has made progress with the development of biodegradable plastics— which can be broken down by environmental microbes in years rather than lasting for centuries. However, they still usually require petroleum to produce. Polylactic acid (PLA) is made from fermented plant starch, usually corn, but this comes with similar issues as previously discussed with corn-based biofuels, as well as biodegrading relatively slowly.
Polyhydroxybutyrates (PHBs) are one promising solution for future plastic needs, as they have similar desirable qualities as other plastics (moldability when heated, for example), they biodegrade well, and they are able to be biosynthesized by microbes. Rather than using a heterotroph like E. coli or yeast as a chassis for PHB synthesis, which would face the same issues of feedstock requirements as it would for biofuel production, autotrophic microbes again seem to be the answer when efficiency and carbon neutrality are the goal.
One chemoautotroph, Ideonella spp. O-1, can grow and produce PHBs using industrial exhaust gases made up of CO, CO2, and H2. However, there is the risk of explosion when working with hydrogen gas, and a system like this would be expensive to operate compared to alternatives. Sulfate-reducing bacteria, such as Desulfonema, Desulfosarcina, and Desulfobacterium can also produce PHBs from precursor molecules (caproate, benzoate) without those downsides, but they grow relatively slowly. As with biofuels, TIE- 1 is an attractive system for PHB production. Its ability to grow using an electrode, as well its ability to acquire energy via photosynthesis, makes TIE-1 a very efficient workhorse for MES toward sustainable bioplastic production.
Microbial Diversity
Something that we’ve seen again and again throughout this eBook is that the first microbe that is discovered expressing a given synthetic pathway is not necessarily the optimal platform for scalability or efficiency. Bacteria capable of EET are frequently anaerobes, preferring or requiring an oxygen-free environment, which can pose problems if the goal is a resilient electrosynthesis bioreactor community. Certain microbes, like Clostridium acetobutylicum, are obligate anaerobes, and are killed by exposure to oxygen. Others, like Shewanella oneidensis, are able to survive oxygen exposure, and this is one of the reasons they have become model species of electroactive microbes studied in the lab. And photosynthetic electrotrophs, such as Rhodopseudomonas palustris, have the added benefit of getting energy from sunlight to improve the energy efficiency of their MES.
By poising an electrode at a certain voltage (such as connecting it to a battery) and placing it in an environment, — a body of water, soil, or anywhere else bacteria like to live— it is possible to isolate novel electrotrophic bacteria. The benefits of this are twofold, as it could identify either a strain that could be a better chassis for a known MES pathway, or it could identify a strain with a new (or more efficient version of a known) biosynthetic pathway. As we move away from fossil fuel-dominated industry, discovering ways to microbially synthesize the numerous organic chemicals historically made from petroleum would do a lot to ease the transition.
Microbes have been on this Earth for orders of magnitude longer than we have, and they have had billions of years to evolve and specialize metabolisms for their environmental situations. Evolutionary processes have produced a huge diversity of microorganisms that are more suited for MES in an industrial setting. It is almost certain that we may isolate novel strains, which have evolved through natural selection to be more suitable for applications like MES compared to the few model organisms we study today. In the Bose Lab, we are constantly isolating new microbes from environmental samples, and we hope that some of these will be future models for climate technologies like MES.