After a stroke, neuroplasticity contributes to spontaneous recovery; exactly how, Professor Francesco Pavone and colleagues are investigating
Cerebral stroke often leaves victims with significant psychical and physical impairments – from vision problems to aphasia and motor deficits. It is the number one cause of adult disability worldwide, and have a huge impact on public health.
The metabolic distress and long-term neuronal cell loss associated with stroke originate from ruptured blood vessels (haemorrhagic stroke) or aggregates of platelets and blood cells that clog cerebral blood vessels (ischemic stroke) and cause a shortage of glucose and oxygen. The destruction process is complex since it originates from cellular damage, evolves to the network level, and eventually extends to connected regions in distal areas.
Only very early intervention (within a few hours) with thrombolysis can dampen ischemic stroke damage. Currently, only about 10 per cent of all stroke patients reach a hospital early enough or fulfil the criteria for receiving thrombolysis in the therapeutic time window. Prognosis and recovery depend mainly on the location and extent of the lesion.
For many years, people have thought of brain wiring as a rigid structure and once injuries such as stroke occurs brain areas and functions are lost forever. This old paradigm of the adult brain as a static structure has recently been replaced by a much more dynamic view where growth, connectivity changes and remodelling can occur after injury and play a crucial role in recovery and functional repair. Stroke patients show various degree of spontaneous recovery weeks to months after the incident.
The pattern of spontaneous recovery
Among the most obvious factors that contribute to the extent of spontaneous recovery are infarct size, infarct location, and age. Most spontaneous recovery tends to occur within the first 3 months. While patients with milder deficits achieve spontaneous recovery more quickly, the pattern of spontaneous recovery can also differ within the same patient for different functions. So far, efforts to summarise this process have been frustrated due to variability across subjects and across neurological domains.
The brain consists of billions of nerve cells and circuits whose rewiring happens at multiple levels, from single cell-to-cell contacts (synapses) to small circuits and functional macro-areas. In order to understand the mechanism of rewiring after stroke – the variability of which eventually leads to very different recovery outcomes – neuronal plasticity after stroke needs to be tackled layer by layer and reorganised through an organic view. Indeed, since neurological disorders affects brain structure and functionality at multiple levels, we believe that a great effort should be directed into coordinating different techniques that can get to different layers. Accordingly, appropriate animal models are needed to investigate stroke and guide the development of effective therapeutic strategies.
My lab focused on the development and combination of different imaging modalities to visualise complementary aspects of brain structure and function. The correlative imaging approach we used gave access to fundamental insight into different spatial and temporal scales of brain functionality. The small though solid bridge it provides between different types of data can be promisingly expanded towards a unified approach covering most perspectives. A great deal of information can be gained on a neurological disease like stroke by following a multi-layer pipeline (depicted in Figure 1). Stroke alters and triggers changes in intra- and inter-hemispheric connectivity; this rewiring aims at compensating for the loss of function.
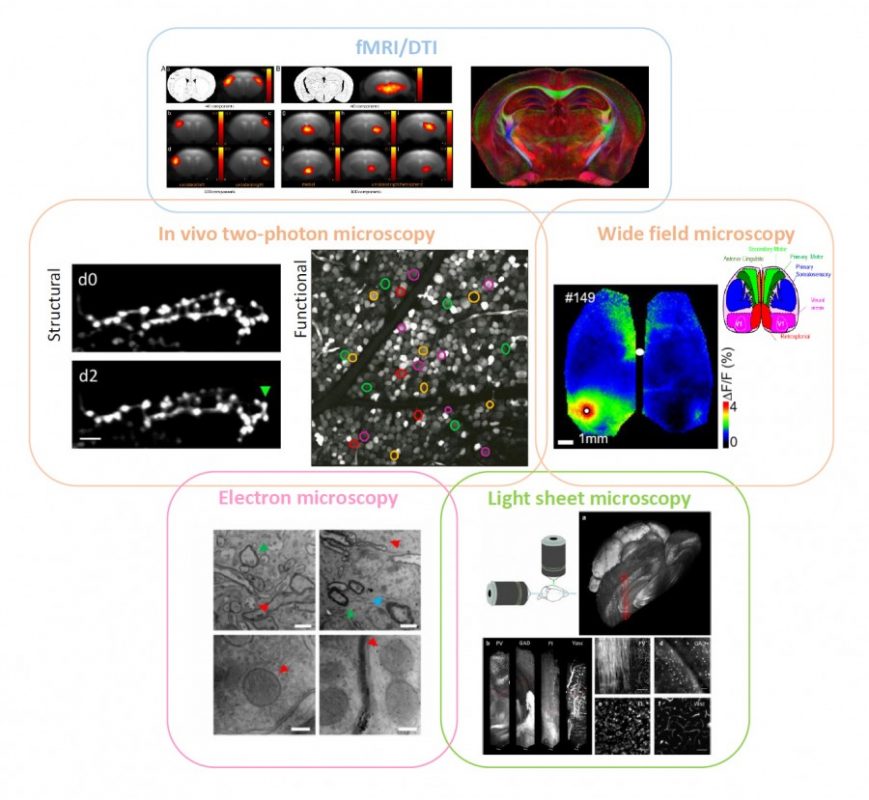
fMRI on (human and) mouse brains affected by stroke can tell the progression of the pathology over time, showing the plastic remapping of distant regions over the whole brain. Magnetic resonance imaging does not have enough resolution to infer what the cellular trigger of this remodelling is; simultaneously-performed two-photon fluorescence imaging of labelled neuronal cells could reveal the structural and functional rewiring underlying fMRI signals in the newly activated cortical area of the same mouse with subcellular detail. Optical imaging on stroke animal models is capable of providing fundamental insight into neuronal remodelling and synaptic plasticity while accurately depicting the functional remapping of the damaged cortex, and revealing angiogenesis and hemodynamic adaptation over time. Alterations in long-range projections underlying inter-hemispheric plasticity can be studied ex vivo on the same brain by applying ad-hoc clearing treatments that make it transparent.
Moreover, stroke-induced expression of several molecules and proteins, like growth-associated factors and inflammatory chemokines, can be addressed with multi-round immunohistochemistry over the entire clarified brain by light-sheet microscopy or similar techniques. Once we have the big picture in terms of long-term dynamics and wide-range remodelling, fine details like the presence of synaptic contacts on regenerated axons are available by electron microscopy on targeted regions of the same sample. Informatics tools to align data at different scales within a common framework (i.e. stereotaxic atlases of murine and human brain) and big data storage facilities need to be further implemented and routinely used. Big transnational research partnerships like the Human Brain Project are working in this direction by “developing the integration and algorithmic reconstruction processes required for high fidelity reconstruction of the mouse brain across all levels of biological organization, from genes to cognition”.
Multidisciplinary facilities are needed
Finally, as described in the European Research Grant I was recently awarded, new optical tools like optogenetics can be used to test and finely tune the activation of specific brain areas. Within this project, we will investigate all-optical brain-to-brain information transfer in a mouse model of stroke to boost more efficient recovery of motor function.
Since a completely integrated correlative approach implies that the same animal should be studied by multi-level analysis on several devices, the possibility of having this wide set of tools near to each other, e.g. in the same campus, is not a negligible issue. Multidisciplinary facilities provided with the above-described imaging devices are an essential requirement for setting up this working strategy. In addition, considering the intrinsic difference between individuals (even between mice of the same strain), a multi-level investigation of the same sample would be extremely beneficial to reduce statistic variability, and to cut the number of animals used in the experiments.
In our opinion, this multi-dimensional hybrid strategy could be extremely useful in the investigation of complex brain diseases like stroke, and would speed up the translation of neurobiology studies to clinical settings. Moreover, the setup of pharmacological treatments would crucially benefit from this multi-level investigation given the multitude of information that can be gained at once. We believe this kind of cross-disciplinary multi-scale study will definitely boost our knowledge of the brain.
Professor Francesco S Pavone
European Laboratory for Non Linear Spectroscopy
University of Florence
Tel: +39 055 457 2480
Please note: this is a commercial profile