How viral vaccines work and why they are effective in curbing human viral disease
Viruses were in existence long before humans inhabited the earth. These nucleic acids are obligate parasites of cellular organisms and are thought to have developed shortly after the appearance of Earth’s earliest life forms. Humans have had a symbiotic relationship with viruses for more than 100,000 years. Much like the “good” bacteria in our gut microbiome, we also have over 100,000 “good” viruses in our bodies that maintain homeostasis and help protect us from harm. Viruses continuously train our immune system to react and defend against potentially dangerous infections. Recent studies suggest that the average human is exposed every day to equal numbers of viruses and bacteria, which means our immune system is continuously creating new antibodies to prevent or limit the extent of infections. In some instances, this continual exposure to viruses results in the incorporation of viral DNA sequences into our genome. Approximately 8% of human genomic DNA is thought to be derived from viruses, and viruses have played a key role in mammalian evolution. For example, syncytin-1, originally a virally encoded protein that integrated into the human genome, is essential for the placenta to form. Occasionally, a viral infection is too virulent (lethal) for our immune systems to defeat. An example is the smallpox virus (Variola major), which is estimated to have killed 300 million people since 1900 with an estimated lethality of 30%.
Medical advances have played a key role in enhancing human survival. Just over 100 years ago, the average life expectancy for males at birth was only 47 years with nearly 30% infant mortality within the first year of life. Public health advances that have significantly increased human lifespan by decades are the result of significantly expanded understanding of microbiology, the development of antibiotics, and the creation of effective vaccines. A primary goal of vaccination is to educate the human immune system to recognize a potentially lethal virus and to reduce the amount of virus in the body upon infection so that the individual is mildly symptomatic or asymptomatic. A second public health objective is to significantly reduce the number of individuals that can transmit the virus to non-vaccinated members of the population.
During the 20th century we discovered how to attenuate (or make less virulent) a live virus, and how to create recombinant vaccines to improve their immune targeting and make them safer and more effective. The development and addition of adjuvants to most vaccines since the 1930s stimulates the immune system to effectively make anti-viral antibodies and long-lived memory T cells that recognize the virus quickly if re-infection occurs. As a result of these medical advances, life expectancy in developed countries has nearly doubled and infant mortality has declined to less than 1%. Highly lethal smallpox has been eliminated. However, the co-existence of viruses, animals, and humans means that the emergence of pathogenic viral infections, such as coronavirus 2 (SARS-CoV-2), remains a constant threat.
Viral infections and the immune response
Viruses enter the body via inhalation, ingestion, sexual contact, and insect bites. Viral infections generally involve mucosal surfaces, such as the respiratory, gastrointestinal, and reproductive tracts, but can also affect other systems such as the nervous system or skin. The outcome of a viral infection is influenced by dosage (how much of the virus an individual is exposed to), viral load (amount of virus produced in the body after infection), route of transmission, and the human host immune response. A number of factors affect immune function to make a host more vulnerable or resistant to infection, including genetics, age, and lifestyle factors (e.g. nutrition, co-existing medical conditions, adverse lifestyle habits).
Viruses are not cellular and are not capable of reproducing on their own. Instead, viruses depend upon the infected person’s cells to complete their life cycles. The simplest viruses consist solely of nucleic acid (RNA or DNA) that encodes a protein that directs the replication of viral RNA when it enters the host cell. All viruses have a protective protein coat (capsid) surrounding the viral genetic material, and some viruses also have an envelope or external membrane. Viruses attach to host cells either through adsorption or via binding to a specific cellular target or receptor. In the case of SARS-CoV-2, the external membrane of the capsid contains a spike protein that binds to the angiotensin converting enzyme 2 (ACE2) receptor on host cells, which enables it to enter. The amount of ACE2 expression determines the human tissues most susceptible to SARS-CoV-2 infection. Once the virus enters the cell, it takes control of the host cell’s machinery to produce copies of itself. New viruses are assembled and exit to repeat the process in adjacent cells. Most human viral infections are asymptomatic and pass unnoticed, but immune activation by viruses can produce fever, chills, weakness, muscle aches, inflammation, and malaise. Signaling molecules in the immune system known as cytokines, produce these symptoms and play an important role in suppressing the viral infection. A balance between pro- and anti-inflammatory/regulatory cytokines is also necessary to ensure an appropriate immune response.
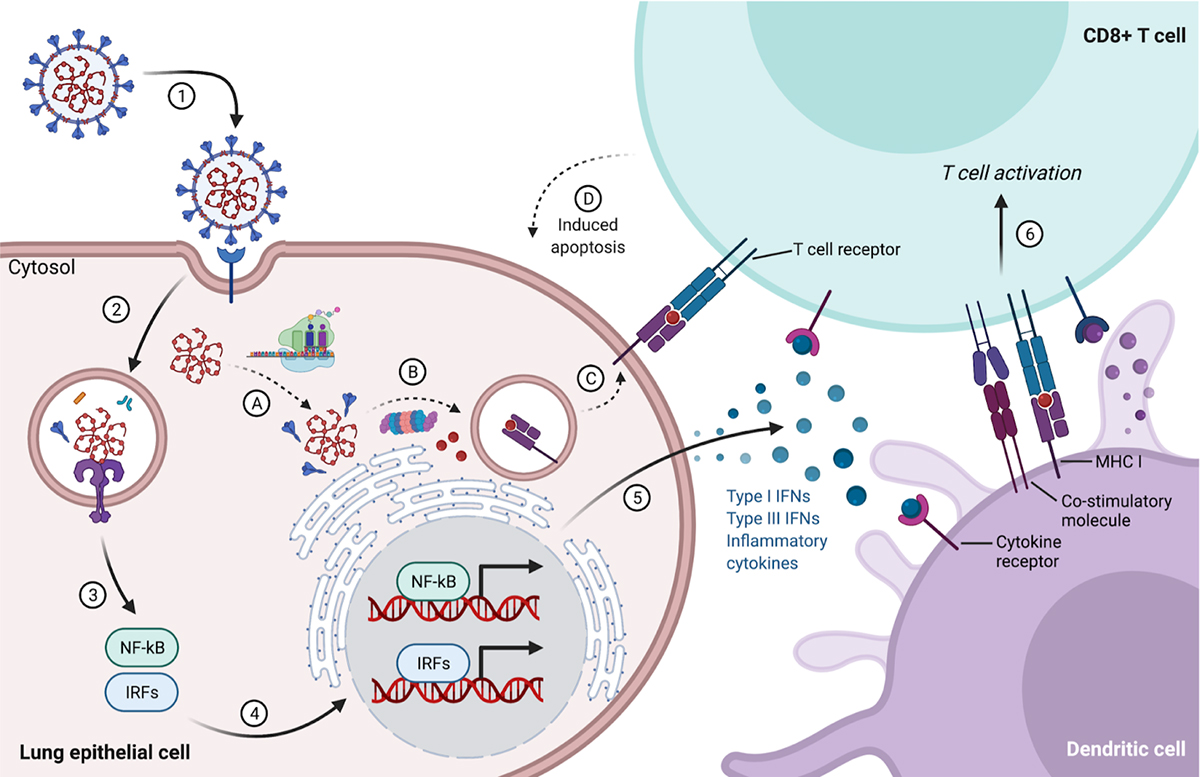
(1) SARS-CoV-2 attaches to a lung epithelial cell via binding to the ACE2 receptor, beginning its life cycle. (2) The virus enters the cell and releases its genetic material (RNA). (3) Innate immune receptors in cellular compartments (endosomes) detect viral RNA, (4) leading to activation and nuclear translocation of cytosolic transcription factors such as nuclear factor-kappa B (NF-kB) and interferon regulatory factors (IRFs). (5) After transcription and translation of the activated host genetic sequences, the cell releases antiviral (interferons – IFNs) and pro-inflammatory cytokines to prime immune cells such as dendritic cells to express co-stimulatory molecules and produce cytokines. (6) Immunogenic dendritic cells sample and display antigens and will activate naive CD8+ T cells that recognize viral peptides bound to MHC class I molecules, leading to T cell proliferation and expansion. Meanwhile, in the cytosol of an infected lung epithelial cell, (A) the viral genome is copied and translated on host ribosomes, making new viral proteins and RNA. (B) Cells are also constantly sampling cytosolic proteins via proteasome degradation, (C) eventually producing peptides for display via MHC I molecules on the cell surface. (D) If the T cell receptor on an activated effector CD8+ T cell binds its cognate antigen, it recognizes the cell as infected and induces apoptosis, a controlled process of cell death, in the target cell.
Figure created with BioRender.com using a modified template (acknowledgement to Gillian Dunphy, PhD).
The immune system must be able to distinguish between infected and uninfected cells to prevent further viral replication while minimizing cellular damage (Figure 1). Foreign viral proteins are broken into small peptide fragments that are bound to class I major histocompatibility complex I proteins (MHC I). The peptide-MHC I complex is displayed on the surface of all nucleated cells and platelets. Cytotoxic CD8+ T cells and natural killer (NK) cells recognize cells expressing viral protein fragments bound to MHC I as foreign and destroy infected cells. Cytokines, specifically type I interferons (IFNs), are important secretory proteins released by infected cells that directly inhibit viral replication and alert surrounding cells to increase surface MHC I molecules, thereby increasing the likelihood of presenting virally-derived peptides to CD8+ T cells and NK cells (Figure 1). Cytokines that contribute to host defense are also synthesized and released by cytotoxic CD8+ T cells and NK cells after contacting infected cells. These cytokines and CD4+ helper T cells provide signals to activate B cells to produce antibodies that recognize viral particles outside of cells and prevent their attachment to host cells. In some cases, as with SARS-CoV-2, a human host’s overactive immune response in the lung can produce excessive cytokine release that inadvertently causes collateral tissue damage, and in extreme instances, organ failure and death.
Viruses use multiple strategies to avoid detection (immune evasion) and in some instances, downregulate the host’s immune response (immunosuppression). Mutation of their genetic code can decrease the likelihood of detection by immune cells (immune escape) or allow the virus to acquire the ability to infect additional host species. Viruses also make proteins that can interfere with host antiviral response. For example, SARS-CoV-2 proteins dampen the type I IFN response, and there is evidence of impaired type I IFN activity in patients with COVID-19. Some viruses, such as varicella-zoster, enter into latency following primary infection, using a dormant, non-infectious form to hide in tissues.
Viral infections: benefits and risks
In most cases, we recover from pathogenic viral infections and develop long-lasting immunity. Adaptive immune cells (B and T cells), which previously encountered a particular virus, are able to respond quickly upon subsequent infections, forming the basis of immune memory. Natural immunity is the most direct benefit to the host following viral infection. Viral infections early in life can be beneficial in “educating” the immune system. As an example, the function of NK cells is enhanced in mice latently infected with a murine herpesvirus as compared with control animals. Mutualistic or commensal viruses, such as bacteriophages found in the linings of mucosal membranes, likely play a protective role in guarding humans against invading bacteria.
On the other hand, there are numerous risks associated with viral infections. Infections can become chronic, a condition characterized by the continued presence of infectious virus with persistent or recurrent disease as seen with AIDS or hepatitis C. Viral disease can be accompanied by severe or lingering symptoms associated with a persistent host immunological response. In some survivors of acute COVID-19, there is evidence of neurological complications stemming from persistent cytokine production (fatigue, brain fog, sensory loss), or autoimmune disorders including Guillain-Barré syndrome. Persistent viral infections can increase risk for other diseases such as cervical cancer following infection with some forms of human papilloma virus (HPV), or secondary bacterial pneumonia after viral respiratory infection. Infections during pregnancy have been associated with preterm birth, and adverse neurodevelop- mental outcomes in the fetus (for example, microcephaly seen with Zika virus). While there are new antiviral drugs for some viral diseases (hepatitis B and C, herpesviruses), generally there are no effective cures for most viral infections. Instead, medications are used to treat or relieve symptoms, and these have their own sets of side effects and complications.
Our history with the principles of vaccination dates back centuries
As previously mentioned, the human immune system is continuously being exposed to viral infections. Thus, vaccination is primarily reserved for those viral infections that can cause a significant degree of morbidity and mortality. The principles underlying vaccination have been recognized and practiced by some societies for centuries. As early as the 10th century, civilizations in China, Africa, and India practiced forms of variolation, a term referring to the practice of small, controlled exposures to live virus to develop immunity. In the 16th century, the practice of variolation gained widespread use as a way to combat smallpox. A person seeking immunity would receive a scratch on the arm and have the puss from a smallpox pustule or dried smallpox scab rubbed onto this superficial wound (this is just one example of a variolation technique). The small amount of viral exposure would produce a modest infection much less severe than the typical respiratory route. After recovery, the individual would subsequently be immune to further smallpox infections. The idea of causing a mild viral infection to prevent a subsequent debilitating or lethal infection led Edward Jenner in 1796 to create what is considered to be the “first” vaccine. Jenner infected individuals with the benign cowpox virus, which is closely related to the smallpox virus, allowing them to become immunologically protected against the highly lethal smallpox. Developed over 300 years ago, the word “vaccine” is derived from the Latin word vaccinus, or “of cows” because of its linkage to cowpox. Highlighted in Table 1 are some common human viral diseases and the vaccines that have been developed to prevent them.
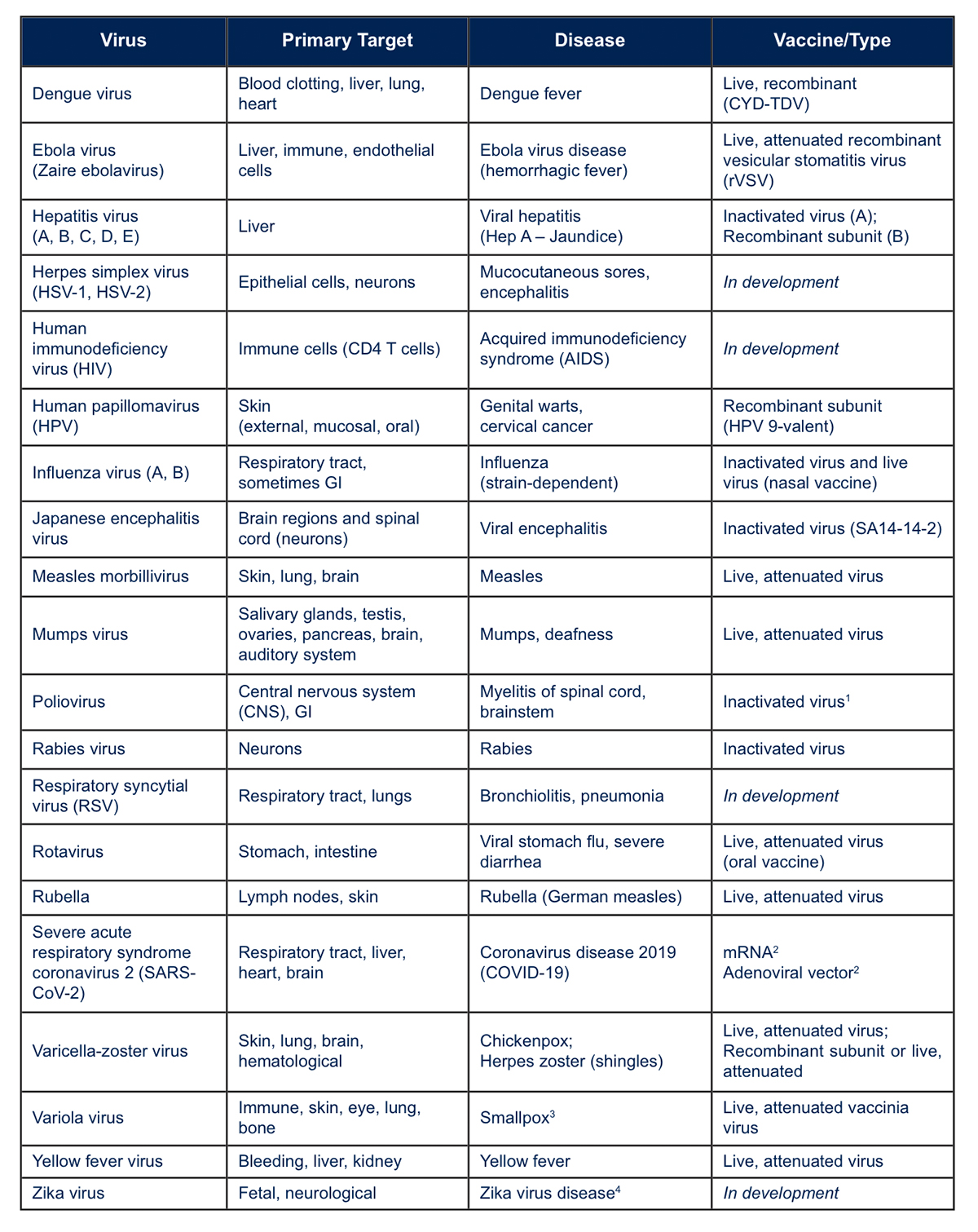
1 – Oral polio vaccine (live virus) is used in other countries. 2 – Approved for Emergency Use Authorization at the time of this writing. 3 – Declared eradicated by the World Health Organization (WHO) in 1980. 4 – Can cause congenital birth defects (microcephaly) in pregnant women who get infected. Main sources of information used to compile this table: https://www.drugs.com/drug-class/viral-vaccines.html, https://www.vaccines.gov/basics/types and CDC and WHO websites.
What are the goals of vaccination today?
People increasingly occupy the same spaces as animals and insects that carry and can transmit novel viruses that are pathogenic to humans. Vaccines developed against these novel pathogenic viruses may not always prevent subsequent disease. However, the goal of effective immunization is to reduce viral replication below the point at which the virus is transmissible or induces a disease state. At this moment, the available data suggests that the Pfizer-BioNTech, Moderna, Johnson & Johnson, and AstraZeneca vaccines developed against SARS-CoV-2 have the capability to reduce viral load in those vaccinated and significantly reduce severe COVID-19 illness and viral transmission.
Immune function and responses to viral insult varies between humans. Most individuals will respond to a viral infection by creating antibodies that recognize short protein fragments of the virus referred to as epitopes. A person’s immune system produces an array of antibodies against a set of viral epitopes, and this array can differ between individuals. As is the case with COVID-19, antibodies that recognize specific viral epitopes permit a more effective immunological response upon subsequent exposures to the virus. Depending upon their design, vaccinations help direct a person’s immune system to produce neutralizing antibodies that are most efficacious in reducing the viral load. Furthermore, vaccines effectively promote the development of memory T and B cells that can rapidly induce production of antibodies upon re-infection. However, in individuals who are immunodeficient or immunocompromised, vaccination will not produce the desired protective immunological response. For these individuals, large scale vaccinations of the population are protective by reducing viral prevalence within the population, thereby decreasing their chances of encountering the virus.
What types of vaccines are used today?
Several types of vaccination strategies are used currently and vaccines commonly used include live-attenuated viruses (a weakened virus), inactivated viruses (dead viruses), and subunit or recombinant subunit (a piece of the virus) (Figure 2). Today, vaccination with attenuated live virus is reserved for populations in which it would be difficult to obtain sufficient large scale vaccination to prevent viral outbreaks. Common ingredients in vaccines include active components, preservatives, and stabilizers (Figure 3). Included in many vaccines are adjuvants that boost a person’s immune response to the vaccine. Aluminum adjuvants have been used since the 1930s and are present in vaccines for hepatitis A, hepatitis B, and Haemophilus influenzae type b. In vaccines composed of intact, dead virus, the glycoproteins associated with the viral capsid acts as an adjuvant. RNA vaccines contain liposomes that encapsulate and protect the RNA, and facilitate cellular entry. These liposomes serve as adjuvants. Adjuvants are not used in the live, viral vaccines in which the goal is to trigger a normal immunological response to weakened strains of replicating viruses.
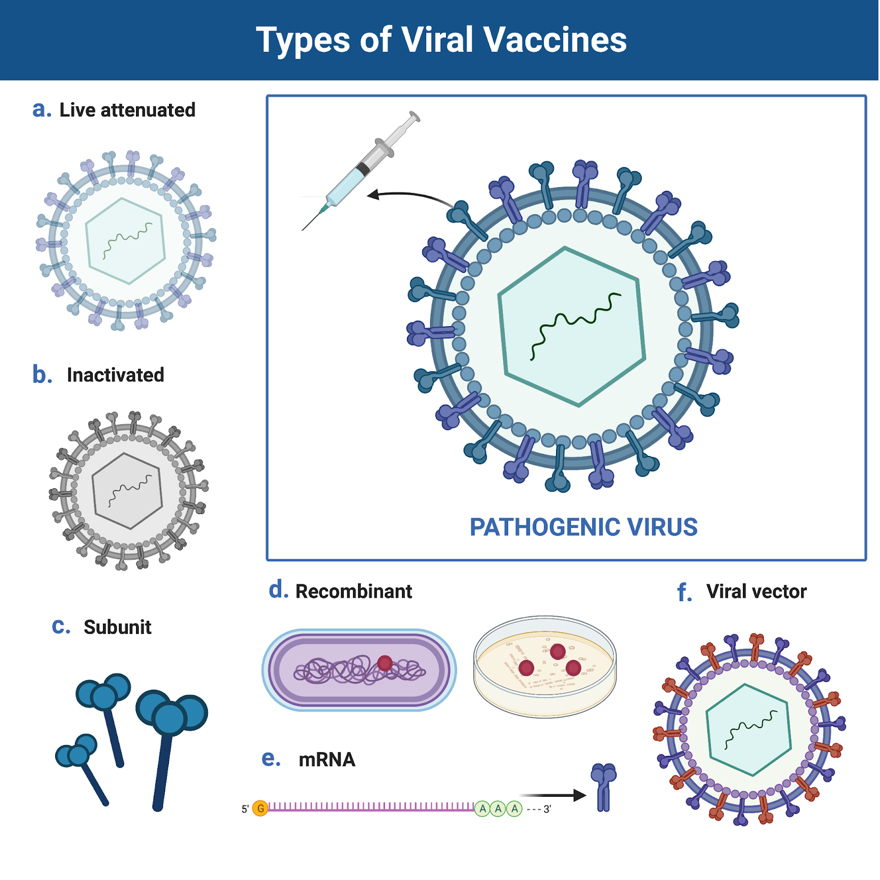
(a) Live, attenuated vaccines such as the MMR vaccine contain viruses that are alive but weakened. This is typically done in a laboratory by continually culturing the virus to the point where it is no longer virulent.
(b) Inactivated vaccines, such as the polio vaccine, contain viruses that are fully dead. This is typically done in a laboratory using heat or chemicals. (c) Subunit vaccines contain just a piece of the virus.
(d) Recombinant vaccines, such as the Hepatitis B vaccine, use genetic engineering technology to produce the active ingredients. Often these techniques are combined, with many viral vaccines consisting of a recombinant subunit (Table 1). These types of vaccines often include adjuvants. Adjuvants are substances added to vaccines in order to increase the immunogenicity of the vaccine. (e) One of the most recent advances in vaccine production is the use of mRNA (messenger RNA) technology. mRNA is a single strand of nucleotides (adenine, uracil, guanine, or cytosine), in this case encoding the instructions for a viral protein that will activate the immune system. (f) Viral vector vaccines employ a modified version of a different, non-pathogenic virus, which serves as a vector to deliver to cells instructions (such as DNA) from a part of the pathogenic virus.
Figure created with BioRender.com using a modified template.
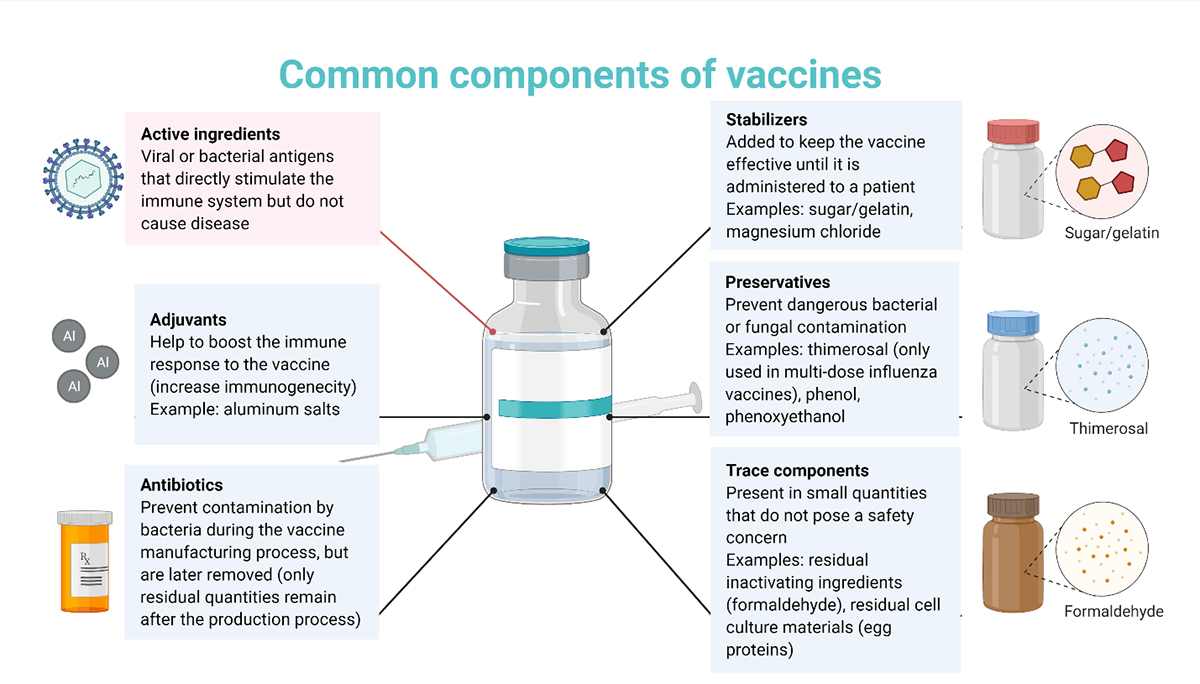
Vaccines contain only essential ingredients each of which has a specific purpose. Ingredients that provide protection (immunity) include the active ingredients and adjuvants. Ingredients that keep the vaccine safe and effective include preservatives and stabilizers. Some ingredients used during the vaccine manufacturing and production processes remain in residual amounts, including antibiotics and other trace components.
Figure created with BioRender.com using a modified template.
The urgency of the global COVID-19 pandemic focused global efforts on the creation of the first mRNA vaccines. In December 2020, mRNA vaccines for the prevention of COVID-19 were approved for use in the United Kingdom (U.K.), followed shortly by an emergency use authorization (EUA) for these vaccines by the Food and Drug Administration (FDA) in the United States (U.S.). At the time of this writing, both Pfizer-BioNTech and Moderna have started the process to move from the EUA distinction to full FDA approval.
While the adaptation of mRNA technology for the use of viral vaccination is relatively new, mRNA technology for therapeutic purposes has been established over the past two decades. In addition, several DNA vaccines for COVID-19 have been approved in the U.K. and U.S. These vaccines utilize a harmless adenovirus vector to deliver the DNA to cells. Types of COVID-19 vaccines currently available in the U.S. and U.K. include the Pfizer-BioNTech (lipid nanoparticle, mRNA), Moderna (lipid nanoparticle, mRNA), Johnson & Johnson (adenovirus, DNA), and AstraZeneca (adenovirus, DNA). In brief, these vaccines work by delivering the instructions (mRNA or DNA) on how to make a small piece of the SARS-CoV-2 virus (specifically a part of the spike protein). The cell will then produce and process the spike protein to display it on the outside of the cell via presentation on MHC molecules. Immune cells that recognize this piece of spike protein as foreign will become activated and expand, resulting in effector T cells and the generation of antibodies from B cells (Figure 4). This allows the body to recognize and mount an immune response to the SARS-CoV-2 virus if it enters the body. As mentioned above, the vaccines do not prevent SARS-CoV-2 from entering the body and possibly replicating, but through the generation of memory cells, it does prevent SARS-CoV-2 from replicating to the point of causing severe illness or death, and significantly lowers the risk of being able to transmit the virus to others.
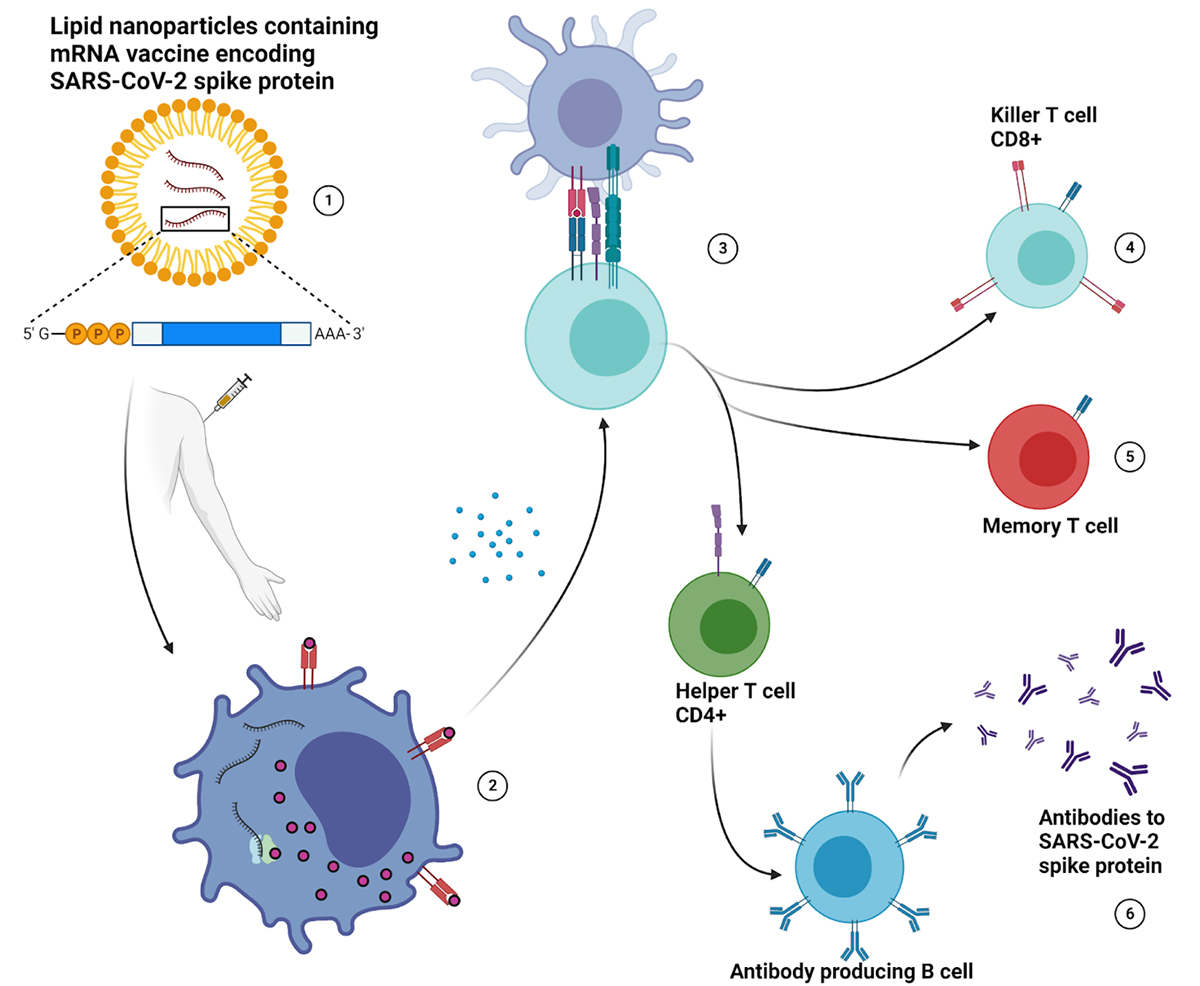
(1) The mRNA sequence required to produce the SARS-CoV-2 spike protein is packaged into lipid nanoparticles, which also act as an adjuvant for the COVID-19 vaccine, then administered to patients via intramuscular injection in the arm. (2) Upon docking to a cell, the packaged mRNA is released into the cytosol, where ribosomes translate the mRNA instructions and produce spike protein (antigen). (3) The subsequent cellular degradation of the spike protein produces viral peptides that are presented on MHC I molecules, displaying antigen on the cell surface. The release of cytokines through activation of antiviral signaling pathways alerts nearby cells to the presence of an “infection”, increasing the probability of naive T cells complexing with activated immune cells that present antigen derived from the SARS-CoV-2 spike protein. The actions of cytokines are also responsible for many of the side effects experienced after vaccination. (4) T cells that recognize peptide antigens from the spike protein become activated, expand, and carry out their effector functions. CD8+ T cells, also known as killer T cells, release additional cytokines locally, and will release cytotoxic granules to induce apoptosis in infected cells. (5) Memory T cells are antigen specific T cells that are easily reactivated and survive for long periods of time after exposure, leading to a more efficient immune response during subsequent infections. (6) CD4+ T cells, also known as helper T cells, activate naive B cells, leading to the production of antibodies that recognize epitopes on the SARS-CoV-2 spike protein. Neutralizing antibodies intercept SARS-CoV-2 and prevent it from binding to host cells, instead targeting it for destruction.
Figure created with BioRender.com.
Risk-benefit analysis in the context of vaccines
We make risk-benefit assessments every day of our lives. Driving your car to work involves weighing the risk associated with automobile travel – your life-time odds of dying in a motor vehicle accident are ~1% – versus the benefits of driving. Most people readily accept the risks of driving to work. This analogy can be translated to actions taken to avoid the outcome of COVID-19 infection. The risk of developing severe illness (defined here as requiring hospitalization and including death) from native COVID-19 infection in unvaccinated adults over 18 is approximately 19%; whereas the risk of severe illness from COVID-19 infection for a fully vaccinated individual is approximately 0.01%.
Whether one’s immune response is stimulated by a native viral infection or under the controlled circumstance of a vaccination, there is some individual risk given the large variance in peoples’ immune response. Although severe allergic reaction to vaccination is rare, some people (including those with a history of severe anaphylaxis) may have different risk for this adverse outcome than the majority of the population. The risk of blood clotting (thrombosis) with thrombocytopenia syndrome (blood clots with low platelets) occurring after receiving the Johnson & Johnson or AstraZeneca vaccine is approximately 7 per 1 million for women ages 18-49, and even lower for those outside that demographic. The risk of developing blood clots from a native COVID-19 infection is about 39 per 1 million, significantly larger than the risk of developing clots from vaccination.
The technology to develop the COVID-19 vaccines had already been in development for quite some time. Although the vaccines currently available in the U.S. were authorized via the FDA’s EUA, this does not mean they were not properly tested. To be authorized for use under the EUA, therapeutics and/or tests must meet extremely strict standards. The clinical trials conducted prior to public use involved thousands of participants, on par with the numbers used for “full” approval. Currently, millions of people have received vaccines for COVID-19, significantly expanding the base of knowledge regarding the efficacy and safety of these vaccines.
Many people have questioned what the “long term” effects of the COVID-19 vaccines will be. It should be noted that viral proteins resulting from any of the vaccination regimens are short-lived. Historically, vaccinated individuals do not “shed” viral proteins. Similarly, viral nucleic acids introduced by vaccination are even more short-lived than viral proteins and they cannot integrate into the human genome. Historically, any adverse effects associated with a vaccine occur within two months of receiving the vaccine (e.g., the low risk of blood clotting after Johnson & Johnson or AstraZeneca vaccines occurred within this time frame). A rare exception to this “two month” window is the possibility of antibody-dependent enhancement of immunity (ADE) following vaccination. ADE occurs when antibodies generated against a viral antigen do not neutralize it, but instead help facilitate viral entry. This unusual condition has been reported in some cases of childhood vaccination against dengue fever. There are multiple strains of dengue and immunological antibodies against one strain may be ineffective in neutralizing the other strains of dengue virus. ADE is hypothesized to have led to the death of 14 children when 800,000 children in the Philippines were vaccinated against all four serotypes of the Dengue virus. In clinical vaccine trials researchers constantly monitor for the possibility of ADE. So far, no instances of ADE have been found associated with COVID-19 vaccines. Common misconceptions about vaccines are addressed in Figure 5.
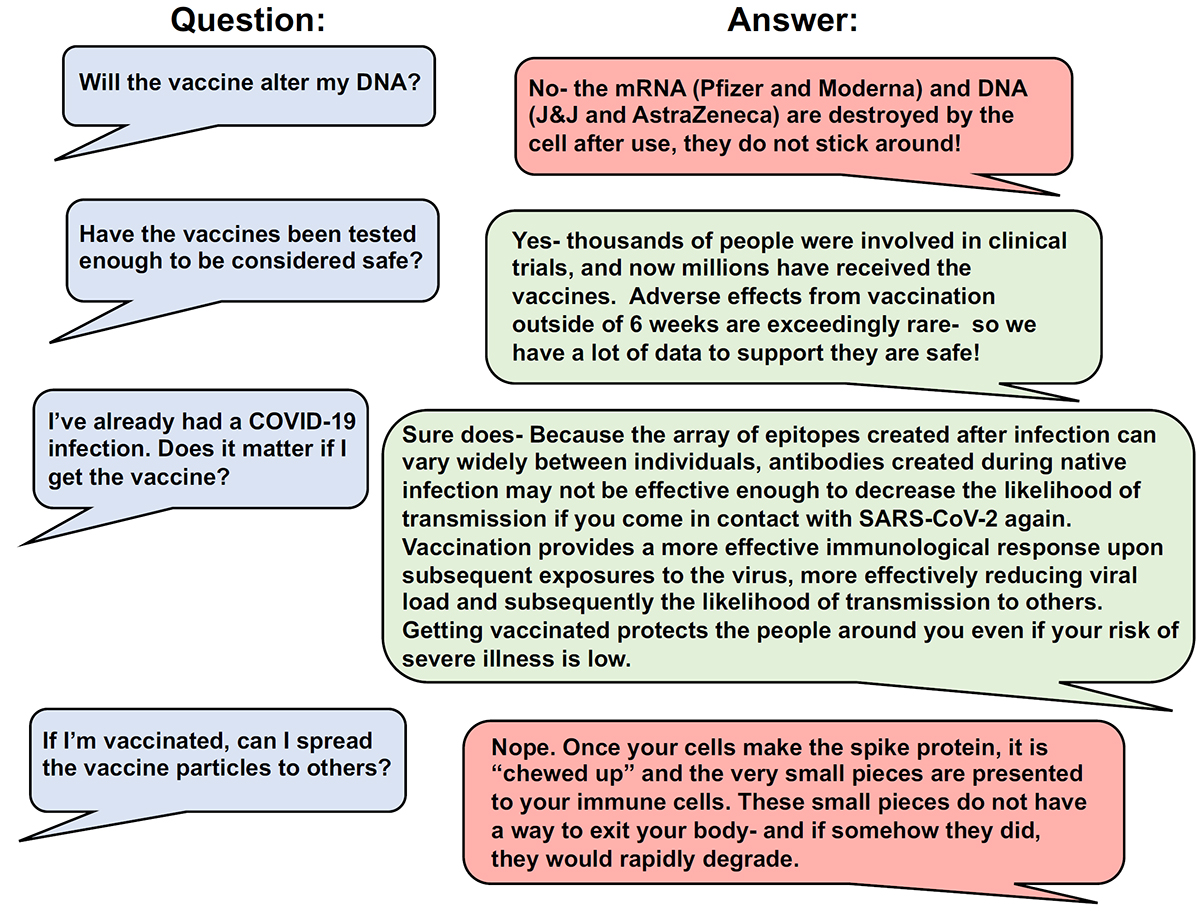
(1) The mRNA sequence required to produce the SARS-CoV-2 spike protein is packaged into lipid nanoparticles, which also act as an adjuvant for the COVID-19 vaccine, then administered to patients via intramuscular injection in the arm. (2) Upon docking to a cell, the packaged mRNA is released into the cytosol, where ribosomes translate the mRNA instructions and produce spike protein (antigen). (3) The subsequent cellular degradation of the spike protein produces viral peptides that are presented on MHC I molecules, displaying antigen on the cell surface. The release of cytokines through activation of antiviral signaling pathways alerts nearby cells to the presence of an “infection”, increasing the probability of naive T cells complexing with activated immune cells that present antigen derived from the SARS-CoV-2 spike protein. The actions of cytokines are also responsible for many of the side effects experienced after vaccination. (4) T cells that recognize peptide antigens from the spike protein become activated, expand, and carry out their effector functions. CD8+ T cells, also known as killer T cells, release additional cytokines locally, and will release cytotoxic granules to induce apoptosis in infected cells. (5) Memory T cells are antigen specific T cells that are easily reactivated and survive for long periods of time after exposure, leading to a more efficient immune response during subsequent infections. (6) CD4+ T cells, also known as helper T cells, activate naive B cells, leading to the production of antibodies that recognize epitopes on the SARS-CoV-2 spike protein. Neutralizing antibodies intercept SARS-CoV-2 and prevent it from binding to host cells, instead targeting it for destruction.
Figure created with BioRender.com.
The path forward
While great efforts have been made to characterize and improve vaccines, there are still many unanswered questions. Particularly within the context of COVID-19, people may wonder how long they will have immunity after getting vaccinated. The good news is that vaccination with Pfizer-BioNTech or Moderna vaccines appears to promote the production of neutralizing antibodies for at least six months. At this time, it is too early to know how far that will extend. Hopefully, these vaccines will elicit the generation of long-lived memory T cells, a type of immune cell that will survive for long periods of time and can provoke a much stronger and faster immune response upon viral re-exposure. Of significance is that patients infected with SARS (a closely related virus to SARS-CoV-2) had memory T cells that persisted for up to two decades after exposure, whereas the memory B cells responsible for antibody generation and their produced antibodies were undetectable after a couple of months. This implies that memory T cells are important for persistent immunity against viral infections. Characterizing the efficacy of the different COVID-19 vaccines against mutated forms of SARS-CoV-2 is still a work in progress with the newer mRNA vaccines directed against the viral attachment site appearing to remain protective. These scenarios likely can be addressed via booster shots in the future, analogous to influenza vaccines. The new mRNA vaccines easily permit sequence adjustment of the mRNA backbone to target viral variants that have small mutations, allowing for rapid development of countermeasures to new or mutated viruses.
With pregnant populations, and more recently children as young as 12 now being approved to receive the COVID-19 vaccines, concerns are starting to dwindle about the safety of these vaccines. All approved vaccines will continue to undergo what is known as post market requirement studies, which forces the companies producing these drugs to continue studying their safety in all populations for many years to come. While it may take that long to fully characterize the safety profile of these vaccines, for now it seems that they are having the desired effect. Invariably, the question will arise: when will life be back to normal? No one can answer that question for sure, but how will we get back to normal? The answer to that question is through the widespread use of safe and effective vaccines.