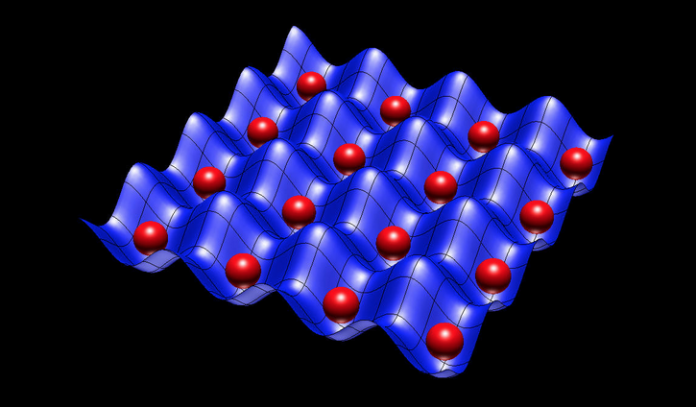
F Barry Dunning and Thomas C Killian from the Department of Physics & Astronomy at Rice University depict the benefits of research into the remarkable physical and chemical properties of Rydberg atoms
Advances in experimental capabilities frequently open up new avenues for basic scientific research that lead to new, and unanticipated, practical applications. One such example is the study of exotic highly excited atomic states, termed Rydberg states after the Swedish spectroscopist J. R. Rydberg who first explored their characteristics. The novel properties of Rydberg states are now being exploited in areas such as sensing, quantum information and quantum simulation.
Normal ground-state atoms comprise a compact, positively charged nucleus surrounded by one or more negatively charged electrons tightly bound by electrostatic forces, resulting in an atomic diameter of a few tenths of a nanometre. If an electron in the atom is given energy it can transition into one of an allowed series of excited states whose energies and orbital motions are defined by the principal quantum number n. As the energy transfer, and n increase, the electron is able to travel farther from the nucleus. The atomic diameter increases as n2 and, for n~1,000 becomes a few tenths of a millimetre. Such high-n Rydberg atoms thus represent giants on the atomic landscape and their size results in very unusual physical and chemical properties. For example, the energy required to strip the excited electron from such atoms, which decreases as 1/n2, is very small, making them not only large, but also extremely fragile. Nonetheless, in atomic terms their lifetimes are long and can amount to milliseconds or more, ample time to allow their study.
The excited electron is so far from the nucleus and the remaining inner electrons that the electrostatic field it experiences from them is very weak. In consequence, its motion can be strongly perturbed, or even dominated, by the presence of small background electric (or magnetic) fields, or by the fields generated by neighbouring Rydberg atoms. Indeed, application of even a modest electric field can be sufficient to tear the excited electron from the atom. Because of this extreme sensitivity to external fields, Rydberg atoms are now being exploited as electric field sensors. Furthermore, since all atoms of a particular species possess identical properties, Rydberg-based electric-field “meters” can be readily reproduced with the knowledge that all will respond identically and share a common calibration.
To measure static electric fields, the changes they induce in the Rydberg energy level structure are observed using laser or microwave spectroscopy, an approach that can detect fields of only a few microvolts per centimetre. Rydberg atoms are also well-suited to detecting oscillating electric fields over a wide portion of the electromagnetic spectrum that spans radio frequencies to the near infrared, with applications in communications, radar, and health care.
Many such applications take advantage of an effect termed electromagnetically induced transparency which involves a cloud of atoms through which is directed a “probe” laser beam tuned to a frequency that the atoms would normally strongly absorb, and a superposed “control” laser beam tuned to excite the atoms to a Rydberg state. With careful choice of the control beam frequency its presence can lead to the transmission of the probe beam through the otherwise opaque medium. Given that Rydberg states are extremely sensitive to external electric fields, their presence can cause marked changes in the probe beam transmission.
Rydberg atoms are so large that in dense gases the electron orbit can enclose many ground-state atoms whose interactions with the electron create a weak chemical bond, forming giant ultralong-range “Rydberg molecules” whose size is comparable to that of the parent Rydberg atoms. Such molecules are not stable at room temperature, but laser cooling techniques now allow production of very cold dense gases, permitting the formation of giant molecules comprising tens to hundreds of “atoms bound within an atom.” The atoms in such a molecule constitute a novel form of “quantum matter” with which to study how many-body interactions lead to collective phenomena, like the formation of “quasi-particles” that play an important role in determining the electronic and magnetic properties of materials.
Rydberg-Rydberg interactions underpin many studies involving quantum simulation and quantum logic devices. Such interactions, which are millions of times stronger than those between ground-state atoms, can be controlled by the choice of the Rydberg states excited and by their physical separations. Much of this work exploits “dipole blockade” in which resonant excitation of one atom to a Rydberg state shifts the energies of neighbouring atoms within some critical “blockade radius,” preventing their subsequent excitation. The use of tightly focused laser beams as “optical tweezers” to trap and position atoms has enabled the realisation of Rydberg quantum gates. These gates feature two atoms positioned within a blockade radius and utilise conditional logic, where excitation of the second atom is governed by excitation of the first. Such gates have proven to be fast, reliable and hold promise in the development of a possible quantum computer.
The use of multiple tweezers, or of “optical lattices” formed by intersecting laser beams, provides the opportunity to assemble ordered arrays of Rydberg atoms whose geometrical arrangements match those found in many thin films and solids. Through their interactions they provide a “quantum simulator” with which to examine the properties of the systems they mimic, especially properties such as high-temperature superconductivity and magnetism that result from collective internal interactions, and that are frequently too complex to simulate even on a modern computer. In addition, by varying the atomic arrangements, a wide variety of “artificial” structures not normally found in nature can be engineered and their properties examined. Furthermore, the effects of defects present in a material can be explored by removing selected atoms from the array and examining the changes that this introduces. Such studies speak to the design and engineering of new
materials with tailored electronic, magnetic, and even thermal properties.
Although once purely a scientific curiosity, giant Rydberg atoms are now finding important applications in many technologically important areas. Continuing research into their remarkable physical and chemical properties promises exciting new discoveries that will herald an even broader range of applications.
*Please note: This is a commercial profile
© 2019. This work is licensed under a CC BY 4.0 license.